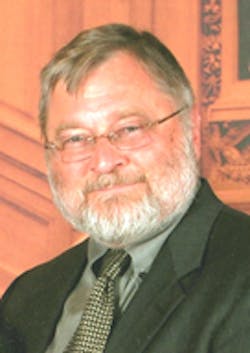
By Eric R. Pearson, CFOS/S/C/T/I; Pearson Technologies
Fiber-optic communications is an amazing and impressive technology. As is obvious, this technology did not arrive fully developed. Rather, through clever and genius-level accomplishments, fiber technology evolved through a series of performance improvements.
To appreciate this impressive evolution, compare single fiber, system capabilities in the mid to late 1970s to those now (Table 1). Today’s capabilities were not possible without the performance improvements presented in this series.This is the first in a series of articles that presents an overview of the evolution of fibers, cables, connectors, splicing, passive devices, and optoelectronics from their initial to current capabilities.
A 5 Step Multimode Fiber Evolution
In this article, we present an overview of the evolution of the optical fiber, with a focus on multimode fiber. Dr. Charles Kao identified the potential for long-distance transmission over glass fiber. In a 1966 paper, he identified the cause of high rate of power loss (attenuation rate) in glass fibers, a significant factor limiting use of fibers for communication. He determined this high rate to be due to impurities in the glass which, if removed, would enable transmission to long distances. This paper marked the start of fiber-optic communications and earned Dr. Kao a Nobel Prize.
In the U.S., Corning Glass Works (now Corning Inc) and AT&T took notice. Corning, a high technology glass company, and AT&T, a communication company, realized the significant synergies between fiber communications and their existing businesses.
Step 1 in the evolution of optical fiber was recognition that fiber could transfer light along its length when the speed of light in the center, the core, is lower than that in the outer region, the cladding (Figure 1). Under this condition, the cladding confines the light to the core by reflection (Figure 2). This is the same mechanism by which we see the sky reflected on the surface of a lake: the speed of light in air is higher than that in water.Step 2 was choosing two glass compositions with different speeds. This choice led to the first fiber type, step index (SI), so called because the speed of light exhibits a step-like change at the core-clad boundary (Figure 1). Sounds simple, doesn’t it?
It is, until one tries to make this fiber. The fiber is formed at 2000° C (3632° F) and cools to room temperature. These two different compositions have different rates of contraction. When the fiber cools from 2000° C, above steel melting temperature, these two regions contract at different rates. If these different rates are not properly balanced, the outside of the fiber has a positive stress. With positive stress on the surface, a minor scratch or other damage can result in a fragile fiber. To understand this, compare a plate which is scratched and bent to a fiber plate that is scratched but not bent. The bent plate can break; the other will not. Like a scratched and bent plate, a fragile fiber would not be a commercially successful product.
In step 3, to avoid fragile fiber, clever scientists and engineers changed the composition of the core by replacing some of the oxide molecules with those that would maintain the difference in speed and result in compression on the surface. This is an example of the subtlties of fiber design: it is design at the molecular level.
Engineers started by choosing simple glass compositions that would produce the desired change in speed of light. These compositions resulted in positive stress on the fiber surface. To the core, scientists added other glasses with reduced molecule sizes to change the surface stress to compression without losing the required difference in speeds. This fiber can be produced without being fragile. But the single composition of the core results in a bandwidth[1] which is insufficient to support adequate transmission distance.
This inadequacy results from several factors, one of which is the light source: it emits rays within an angle to the fiber axis. As a result of this angle, different rays travel different paths through the core (Figure 2), because light travels in a straight path, unless a condition in the path changes. Different paths result in the label for this fiber type: multimode, which indicates that different rays of light can take multiple paths through the core.
These different paths result in multiple path lengths, which, in turn, result in multiple arrival times at the fiber end. Multiple arrival times result in a continuous broadening or spreading of the optical pulse as it moves through fiber. The technical term for such spreading is dispersion. The dispersion in Figure 2 is the largest of five types.When excessive, pulse spreading causes sequential pulses to interfere with one another, limiting bandwidth and transmission distance. Because of different path lengths, dispersion in SI fiber results in bandwidth and transmission distance insufficient for the desired communication use.
In step 3, the clever scientists replaced the single core composition with multiple compositions. This fiber is known as a graded index, or GI, fiber, because the speed of light changes, or is graded, from the center to the cladding (Figure 3).
The composition in the center of the core has the lowest speed of light. Around this center composition, the engineers added multiple compositions. In each subsequent composition, the speed of light increased. Thus, as rays of light move further away from the center of the fiber and experience increased path length, they experience successively increasing speeds of light. Compensation of the different path lengths results.
With multiple compositions, rays of light that travel longer paths travel at higher average speeds. This increased speed reduces the difference in time of arrival of rays with increased path length, resulting in fiber with bandwidth and transmission distance adequate for the desired communication use.
A simple way to understand this compensation is to consider only two rays of light, one traveling parallel to the fiber axis and one traveling at a maximum angle to the axis. The ray traveling parallel to the axis travels the shortest distance at the lowest speed. The ray traveling at a maximum angle travels the longest distance at the highest average speed.
This GI fiber is known as standard GI, OM1 and OM2, depending on the core diameter. The number of compositions began at ~100 and is now up to 2500, all within the 25µ core radius. Again, this sounds simple, doesn’t it?
It is, until one tries to make this fiber. This fiber is not simple to make, for at least two reasons. The first reason is the need to maintain each of the 2500 constant along the fiber length. With 2500 compositions, the thickness of each composition layer is 0.01µ, which is 1/7500th the nominal thickness of human hair!
In addition, the thickness of each composition must be constant along the entire fiber length. Fiber length began at ~ 5 km and is now at least 1500 km, with fiber lengths up to 7500 km. To appreciate this accomplishment of engineers and scientists, consider the sophistication of fiber process control required to maintain constant composition and constant layer thickness at 0.01µ for 1,500,000 m (4,920,000 ft)!
The second reason that this fiber is not simple to produce is the need to maintain a compressive stress state on the surface of the cladding. Earlier, we learned that each chemical composition has a different rate of thermal contraction. Each of these compositions, up to 2500, must be chosen so that the surface is compressed relative to the core. With this stress state, the fiber will not be fragile.
But these adjustments must be done to simultaneously maintain surface compression and path length compensation. You can begin to see the complexity of designing and producing such a fiber: adjust the composition to maintain compensation and adjust it again to maintain the compression on the surface. Repeat, as necessary.
From the late 1970s until the mid 1990s, GI fiber met distance and bandwidth requirements up to a bit rate of 100 Mbits/sec. As bandwidth needs increased, 100 Mbits/sec became inadequate.
This inadequacy was due to the light source used on GI fibers. These light sources, light emitting diodes (LEDs), were inadequate for bit rates at and above 1000 Mbits/sec, the next transmission goal. For this higher bandwidth, fiber transmission needed a different type of light source. This type was the vertical cavity surface emitting laser (VCSEL).
A significant difference between LEDs and VCSELs is the diameter of the area that emits light. This area, the spot size, is 50µ - 200µ for LEDs; 30µ, for VCSELs (Figure 4). The small VCSEL spot diameter results in most of the light staying in the center of the fiber, resulting in a reduction of path length differences (dispersion).Prior to the advent of VCSELs, the GI fiber was graded across the entire core diameter, typically 50µ to compensate for path length differences. Such grading results in grading near the center that was insufficiently precise to enable the VCSEL to transmit to an acceptable distance.
This insufficiency led to a need to grade the multimode fiber near the center in a way to increase transmission distance at bandwidths at and above 1000 Mbits/sec. A fiber graded in this manner is known as laser optimized (LO). Eventually, fiber manufacturers produced three grades of LO fiber: OM3, OM4, and OM5. These fibers enabled transmission as presented in Table 2.
At this stage in fiber evolution, fiber had the multimode capabilities just listed. While VCSEL development stalled at 10 Gbits/sec, transmission requirements continued to increase to 40 and 100 Gbits/sec. Did this increase spell the end of multimode fiber? Scientists and engineers were far too clever to allow such an end.
In step 4, the clever scientists and engineers developed a solution to enable continued use of multimode fiber at transmission rates above 10 Gbits/sec: parallel transmission. In parallel transmission, signals greater than 10 Gbits/sec were de-multiplexed into parallel 10-Gbit/sec paths, hence the term. The de-multiplexed signals were transmitted on separate fibers, with the signals multiplexed at the receiver. Instead of one pair of fibers, one each for transmission and reception, 40 Gbits/sec requires 4 pairs; 100 Gbits/sec, 10 pairs.
The motivation for parallel transmission was avoidance of increased cost from changing to singlemode transmission. Singlemode transmission was significantly more expensive than multimode. An example of this is evident in Gigabit Ethernet transceivers: at one time, multimode transceivers were ~$70/end; singlemode, over $300.
Clever? Definitely. But parallel transmission has at least two disadvantages. The first disadvantage is increased complexity. Instead of one pair per transmission path, 40 Gbits/sec requires 4; 100 Gbits/sec requires 10. Maintaining polarity, that is, avoiding misconnection, with 8 or 20 fibers is more complex than with two.
The second disadvantage is consumption of cable tray volume: parallel transmission cables are larger than two-fiber cables, with two consequences: cable trays fill quickly and/or trays require increased size and cost. Minimizing tray size and cost are important goals in the design of data centers. Thus, though parallel transmission extended use of multimode fiber, it had disadvantages.
In step 5, engineers addressed the disadvantages of parallel transmission. They designed an LO fiber that would enable transmission of 40 or 100 Gbits/sec over one fiber pair, instead of 4 or 10, respectively. This fiber, designated OM5, allowed transmission of 4 moderately spaced wavelengths over the same fiber. Transmission of multiple, moderately spaced wavelengths is known as coarse wavelength division multiplexing (CWDM).
With VCSELs transmitting 4 wavelengths at 10 Gbits/sec, 40-Gbit/sec transmission over 1 fiber pair was possible. When VCSELs evolved to operate at 25 Gbits/sec, 100-Gbit/sec transmission over 1 fiber pair was possible.
Summary
The ever-increasing demand for bandwidth resulted in a 5-step evolution (Table 3).
When increasing demand exceeded fiber capability, the clever and creative, engineers and scientists improved fiber performance to meet this demand. Without any doubt in this author’s mind, their accomplishments have been amazing and impressive. To say that some of these accomplishments were genius-level would be understatement.What’s next?
Demand for increasing bandwidth continued, creating a need for fiber that enabled bandwidth and transmission distance above what multimode fiber could support. That fiber is singlemode, the subject of the article 2 in this series.
For 43 years, Eric R Pearson, CFOS, has been active in fiber communications. For 41 of those years, Pearson Technologies Inc. has provided fiber training in installation and design, and technical consulting, including support in patent and damage lawsuits. Contact Pearson Technologies at: www.ptnowire.com, [email protected], or 770-490-9991.
[1] Bandwidth is the commonly used term. However, bandwidth infers analog transmission. Bit rate infers digital transmission and is the technically correct term. This author follows common usage.